Energy Storage 101
This content is intended to provide an introductory overview to the industry drivers of energy storage, energy storage technologies, economics, and integration and deployment considerations. ES 101 may be helpful for bringing new stakeholders up to speed on the energy storage landscape.
The content is based on EPRI's Energy Storage 101 training courses. We will continue to build out the content with up-to-date content. If you have any suggestions, please email Erin Minear.
Drivers for Energy Storage
There are various factors and forces that are currently driving the adoption of energy storage and influencing the current energy storage landscape throughout the world. Since 2018, the size and duration of projects has generally increased. Announcements for new battery energy storage sites planned over the next 2-3 years have grown — now, individual sites may host hundreds of megawatts and nearly a gigawatt-hour each. By the end of 2018, battery energy storage had been deployed in nearly every region of the U.S. under a variety of ownership models. IPPs owned most of the power capacity, providing market services for ISOs like PJM and ERCOT. Conversely, IOUs owned most of the energy capacity, serving needs such as renewable firming and load shifting in places like California and New England. [1]
Decreases in Technology Costs
Massive research and development investment and manufacturing scale-up has driven costs down for lithium ion battery storage. This was initially driven by the consumer electronics market (e.g. cell phones and laptops) and more recently accelerated by the electric vehicle market. There has been an almost 90% reduction in $/kWh cost in the last decade and lithium ion costs are expected to continue to decrease with additional manufacturing improvements and economies of scale. Solar and wind technology cost reductions are also driving deployment of energy storage for hybrid applications. Bloomberg New Energy Finance projects 2030 lithium ion pack costs at $62/kWh based on observed prices and an 18% learning rate.[4]
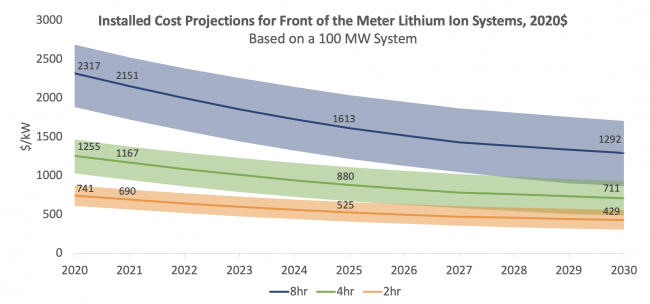
Increasing Renewable Generation
Solar photovoltaic (PV) is driving midday over generation and increased evening ramping requirements which provides a value stream for flexible energy storage. As more solar comes online, the effective net load in the middle of the day decreases. Similarly, wind energy is also driving flexibility needs.
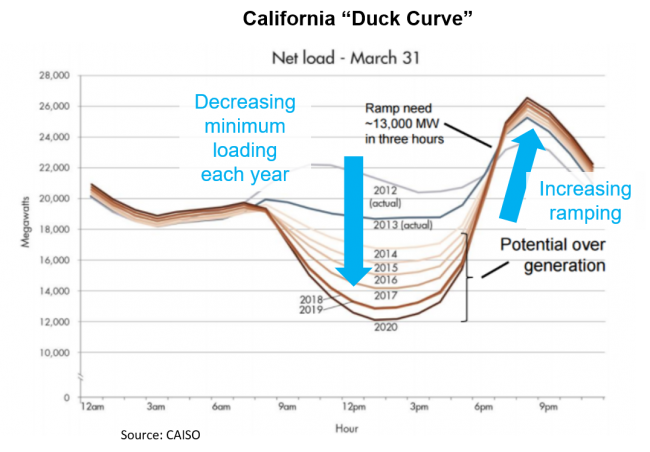
Evolving Utility Needs
- The grid infrastructure (generation, transmission, and distribution) is sized for infrequent peak needs and therefore most assets are under-utilized most of the time. Energy storage can support peak load reduction to provide significant cost reduction opportunity to electricity customers.
- Utility asset infrastructure is aging and peak load reduction may extend asset life and offer opportunity to consider investment in new technologies.
- Peaker plants are only used a fraction of hours per year and energy storage is being considered as peaking capacity in generation planning. Battery storage is already being deployed for this application and as costs decrease they may be cost competitive with combustion turbines in the next decade. When accounting for operational benefits, the crossover point on cost may be sooner.
Increasing Utility Customer Choice and Engagement
Customers (residential, commercial, industrial) are considering energy storage for:
- Bill savings
- Increased energy independence
- Renewable energy goals
- Backup premise or critical loads
Policy and Regulatory Changes
U.S. Federal Policy
In the last decade there has been a shift in policy towards energy storage. At the federal level, FERC has issued several orders as outline below to support energy storage in markets.
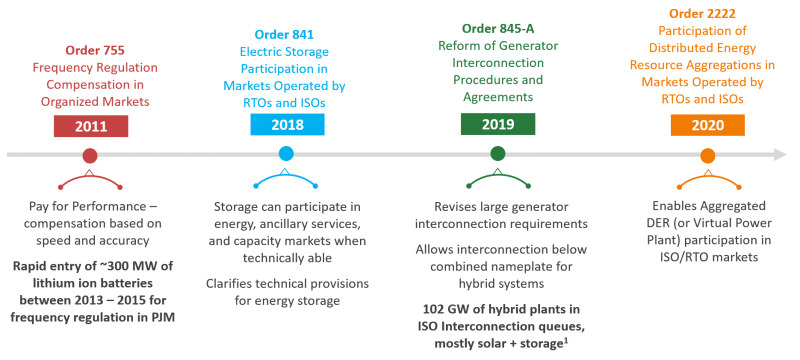
1Lawrence Berkeley National Lab, Hybrid Power Plants: Status of Installed and Proposed Projects.[7]
U.S. State Policy
At the state level, there has been an expanding number of policies to address energy storage in various ways.
- Clean Energy Goals: Carbon-free, renewable portfolio standards, and net-zero goals.
- Procurement Targets: Regulators or legislators set procurement goals and mandates requiring utilities to directly procure or contract storage.
- Resource Plans: State agencies or regulators fund studies or direct utilities to create an energy plan with consideration of storage. Many utilities and states included storage in their resource plan even if not directed to by regulators (not shown on the figure).
- Incentives: Legislators created economic incentives (e.g., rebates or subsidies) for deploying storage.
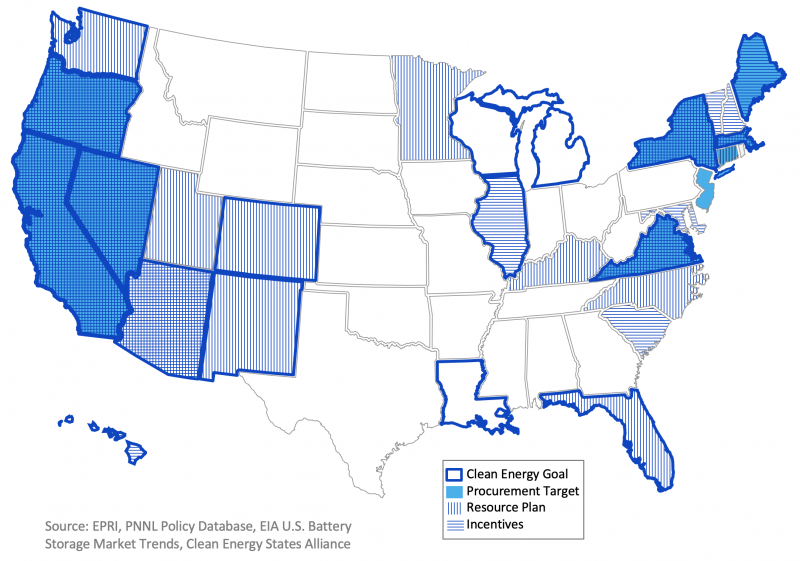
Energy Storage Economics
An economic analysis of energy storage systems should clearly articulate what major components are included in the scope of cost. The schematic below shows the major components of an energy storage system. System components consist of batteries, power conversion system, transformer, switchgear, and monitoring and control. A proper economic analysis identifies the costs associated with each of these components.
Introduction to Grid Services
The economics of energy storage is reliant on the services and markets that exist on the electrical grid which energy storage can participate in. These value streams differ by region, electrical system, and grid domain (i.e. transmission, distribution, customer-sited).
Regardless of the situation, at a high level, energy storage can be utilized across the grid in the following ways:
- Capacity Resource: On the electric grid, capacity is synonymous with power, and to be a capacity resource is to provide power that is reliable and firm, so that it can be dispatched when needed. For example, energy storage can charge itself during times when there's excess capacity and essentially become a capacity resource for times when load is peaking in a particular location or region.
- Flexibility Resource: Energy storage has the ability to increase (charge), or decrease (discharge), the total load on the grid at different times very quickly. This characteristic can be leveraged to decrease ramp rate requirements and stresses on traditional assets during times when high generation ramp rates are required. For example, in the early afternoon when grid load demand is low and renewable generation is peaking, traditional generators must quickly ramp down to prevent over-generation on the system. During this time, energy storage can charge itself with excess renewable energy and discharge the energy later in the afternoon, as renewables ramp down and load demand increases.
- Backup Resource: Energy storage can act as a reservoir for energy which can be saved and used when it is needed. If electric power service is disrupted and energy storage is connected to a critical load, the load can use the energy reserve to ride out the disruption.
- Power Quality Resource: Energy storage can be used to affect the voltage or the VARs at a particular point on the grid. This can be accomplished by energy storage systems that have inverters that are capable of "4 quadrant" operation. This functionality enables the inverter and storage system to either produce or absorb VARs which can affect the voltage voltage around the point of interconnection.
Transmission-Connected and Bulk Energy Storage
The figure below provides a list of the services that energy storage can provide at the transmission or bulk energy storage level (generally 10MW or more). These include generation capacity (sometimes called resource adequacy), flexible capacity (sometimes called flexible resource adequacy), virtual transmission capacity (support transmission reliability or alleviate congestion), energy time shift, ancillary services, black start, and frequency response. This section provides more detail.
Generation Capacity (Resource Adequacy)
Generation capacity is the capability and availability of a resource to contribute to system load needs. Capacity resources are typically committed months to years in advance. As demand and resource mixes change, including generator retirements, new resources are needed to ensure capacity needs are met during all hours and conditions. Assuming sufficient storage duration and reliable response times, energy storage may provide reliable load peak reduction. However, energy storage is more challenging to model and assess than traditional generation, because of its limited energy capacity.
Flexible capacity
As more renewables are deployed, daily fluctuations (i.e. ramp rate requirements) in traditional energy generation are increasing. Grid planners must consider these ramp rates in long-term generation resource planning. Energy storage can alleviate ramp rate requirements by absorbing or releasing energy to effectively reduce the maximum ramp rate required by generators.
Virtual transmission capacity (reliability/congestion)
Circuit load growth downstream of transmission assets drives the need for transmission upgrade investments to ensure reliability in both normal and contingency situations. If appropriately sized and placed on the transmission system, energy storage can reliably reduce peak load below capacity threshold by charging during low load times and discharging to serve loads when the threat of transmission system overload arises. With this strategy, utilities may delay traditional transmission upgrade investments for several years resulting in time-value of money savings and rate-payer benefits.
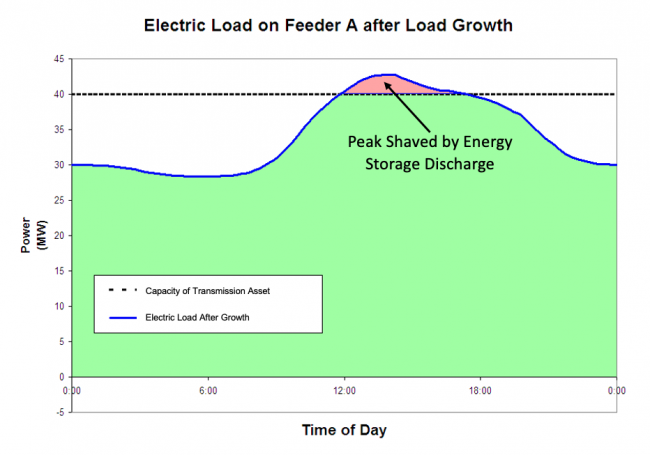
Energy time-shifting
Energy time-shift recognizes an opportunity to economically move energy demand through time taking advantage of fluctuations in the marginal cost of electricity production. To do this, storage can charge when the price of electricity is low (or even negative) and discharge when prices are high. The value of energy time-shift depends heavily on market structure, load growth, and generation mix.
Ancillary services
Ancillary Services are reserve services necessary to support the transmission of electric power from seller to purchaser, given reliability obligations within control areas. The electricity grid operates as a just-in-time delivery system and system operators are responsible for managing voltage, frequency, and system restoration. Variable generation sources such as wind and solar tend to increase uncertainty and variability on the grid. Energy storage, as a flexible resource, can provide instantaneous reserves to balance the grid and leverage it's fast-acting power electronics to perform services like Volt/VAR regulation.
Common ancillary services include:
- Frequency Regulation
- Spinning Reserve
- Non-Spinning Reserve
- Frequency Response
- Black Start
- Voltage/VAR Regulation
Distribution-Connected Energy Storage
The figure below provides a list of the services that energy storage can provide at the distribution level (generally in the 10kW-10MW range). These include virtual distribution capacity, power quality support, resilience / backup power for microgrids, as well as the possibility to provide transmission support in certain scenarios.
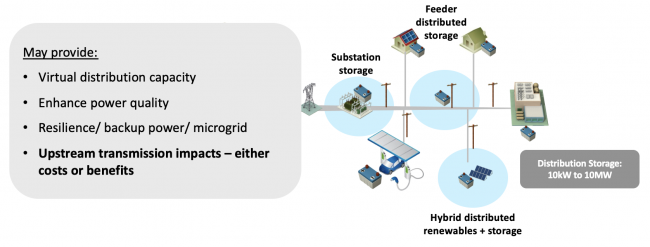
Virtual distribution capacity
This service is similar to the "Virtual transmission capacity", just at the distribution level. In the example depicted below, some time in the near future, more load (homes) will exist on the distribution feeder than the feeder's transformer can handle during peak times. Distribution planners can either upgrade that transformer now, or install an energy storage system downstream of the transformer to ensure its limit is not exceeded for a few more years. In some cases, this energy storage purchase and traditional asset upgrade deferral can provide favorable economics. It is also possible for distribution-connected energy storage systems to provide similar virtual services to the upstream transmission network.
Customer-Sited Energy Storage
The figure below provides a list of the services that energy storage can provide at the customer-sited level (generally in the 2kW-2MW range). These include customer bill savings, power quality enhancements, resilience / backup power for microgrids, as well as the possibility to provide transmission and/or distribution support in certain scenarios.
Value Stacking
Each grid service performed by a battery may provide some economic value, or benefit, but one service alone may not provide enough value to overcome the lifetime costs of the installation. If a specific scenario allows it, it may be possible to approach multiple grid services with one project to improve a projects economics. Keep in mind, there may be regulatory or technical complications that prevent the a project from attempting multiple stacked services.
Understanding Service Compatibility
When value stacking, energy storage service compatibility only flows from bottom up; customer storage may provide distribution and transmission-level services, but transmission storage can NOT provide distribution or customer services.
Reliability vs. Economic Services Reliability services take priority over economic services. For example transmission / distribution deferral services are higher priority than market services.
Local vs. System Level In general, local objectives supersede system objectives when there is a conflict. For example, transmission / distribution deferral is a higher priority than resource adequacy, though operations should be designed such that reliability services never conflict.
Cost Components and Trends
In addition to identification of major components, there are key BESS services that occur throughout the system's lifetime that need to be accounted for in an economic analysis. The left side of the graphic below shows the beginning of life stacked costs for battery energy storage systems. As shown in the owner's upfront costs, the largest upfront cost is the battery itself. One important consideration, given the significant battery costs, is the sizing assumptions that are used for cost estimates. Often, only a fraction of the procured energy is actually delivered to the customer and some fraction is reserved for safety and performance margins, degradation overbuild, AC/DC losses, or is electrochemically inaccessible. Additionally, the graphic shows relative costs for services throughout BESS operation. These services include operating expenses, fixed system maintenance, and decommissioning and end of life services.
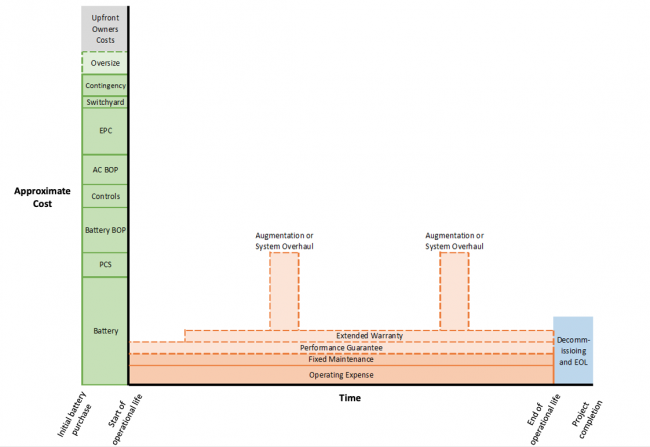
Other generation resource costs are often communicated on a $/kW basis, but energy storage has both power and energy components and the normalized cost will vary with duration. This is highlighted in two examples below where it's clear that the longer duration system has a higher cost per kW of power.
The image below shows lithium ion cost projections from a 2020 EPRI cost study. As demonstrated in the two examples above, the longer duration systems, as of 2020, are more expensive per kW than the shorter duration systems. However, EPRI's cost study projects that the cost per kW of 8 hour duration lithium ion systems is decreasing at a faster rate than 2 hour lithium ion systems.
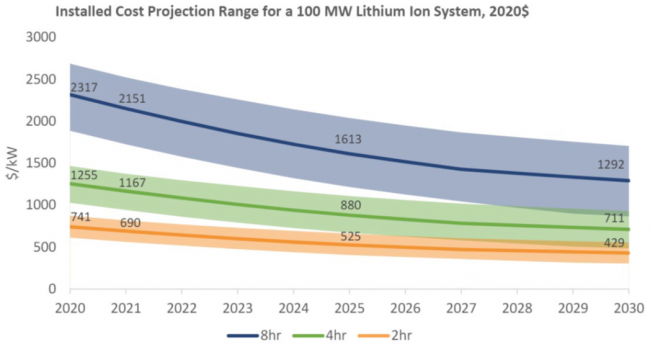
Modeling Energy Storage
Advanced energy storage is a difficult technology to model owing to its limited energy capacity. Operating an energy storage system now can limit its ability to operate in the future. Additionally, energy storage is not yet a common grid asset and is subject to rapidly changing rules, regulations, and standards. But, the flexibility of energy storage can provide a lot of value when operated well and with consideration for the degradation of the system owing to their ability to go from charging to discharging and vice versa quickly and without interruption. Typically, energy storage systems do not serve just one application and instead leverage their flexibility across several stacked services. Service stacking presents additional modeling challenges, though, because the requirements imposed by each service need to be meet in parallel. The need to ensure a storage system can provide stacked services without running out of stored energy typically necessitates detailed, site-specific time series modeling.
EPRI has developed various tools and resources to support the industry's efforts in valuation modeling, some of which are highlighted below:
Resource | Access Level |
---|---|
EPRI Solar Plus Storage Cost Assessment and Design Considerations: Executive Summary (2019) | Publicly Available |
EPRI Energy Storage Technology and Cost Assessment: Executive Summary (2018) | Publicly Available |
ESIC Energy Storage Cost Tool and Template | Publicly Available |
Energy Storage Valuation in California: Policy Planning and Market Information Relevant to the StorageVET Model | Publicly Available |
Energy Storage Technologies
People often think of grid energy storage as electricity in / electricity out with some energy loss in between due to inefficiencies. A more inclusive "energy storage" definition should include technological nuances like supplemental energy sources (e.g. input fuels or heat injection). One must also consider that energy storage systems can output non-electrical energy in the form of heat, cooling, or fuel sources (e.g. hydrogen).
Technical Characteristics of Energy Storage
The specifics of a project's use case(s) will dictate the optimal system attributes. Understanding these attributes, and the trade-offs between them, will help with the selection of a specific technology. For an exhaustive list of considerations, refer to the ESIC Technical Specification Template.
Power, Energy, and Duration
Some of the most fundamental energy storage attributes are power (measured in Watts) and energy (measured in Watt-Hours). Energy storage power is usually provided in kilowatts (kW), megawatts (MW), or gigawatts (GW), while energy is the integral of power over time, so measured in kilowatt-hours (kWh), megawatts-hours (MWh), or gigawatts-hours (GWh), depending on the scale of the system. Sometimes, a duration (hours) is specified instead of energy, this is calculated by dividing the energy (watt-hours) by the power (watts) to return hours. This provides an estimate for the amount of time a system could operate at it's rated power, though in reality, the true time a system takes to charge or discharge is dependent on many factors or limitations.
Relationship Between Technologies and Their Ideal Applications
Different technologies have their own ideal energy-to-power ratio (i.e. duration) which makes them suitable for specific classes of use cases. The figure below breaks the duration domain (x-axis) into 4 different use case bins with common technologies added to the areas that they tend to perform best. Some energy storage technologies, like super-capacitors, are best at responding quickly and come in small modular form factors making them ideal for fast, "power" services like frequency response. Other technologies like pumped hydro are only feasible on a large scale, so are best suited for high "energy" services like energy time shifting.
Key Storage Technology Attributes for Project Evaluation
There are many technology attributes that are most important when performing site-specific project evaluations. So, what technology you ultimately decide to deploy will be a function of what is required for a specific use case. These attributes include: safety, expected operational life, deployment timeline, performance, technology maturity, siting considerations, lifecycle costs, and environmental or public health considerations.
Performance Attributes
This section will describe a few of the many energy storage performance attributes that should be considered. For a more exhaustive resource please visit the ESIC Energy Storage Test Manual, it is free to the public.
Efficiency: Ratio of the delivered discharge energy to the delivered charge energy, including facility parasitic loads. Many factors impact a system's measured efficiency:
- The specifics of the efficiency measurement and calculation
- Location of measurement (e.g. at the DC output of the battery or AC output of the inverters)
- Auxiliary or parasitic load losses
- Power level of a given cycle
- The weather / season
Response Time and Ramp Rate: Some energy storage technologies are able to respond quickly to dynamic control signals while others require more time to ramp up and respond with accurate output. Fast acting energy storage systems may perform dynamic grid services (like frequency regulation) better than conventional alternatives.
Operational Life
The operational life of an energy storage system is a tricky concept to define generally, but it typically refers to how long a system is able to operate before degradation prevents the system from safely and reliably performing its objectives. Different technologies will have drastically different degradation time frames and mechanisms, but most degradation impacts can be described by cycle life or calendar life
Cycle Life: Number of times the energy storage system can be charged and discharged before degrading beyond application requirement. Often, factors like each cycle's depth-of-discharge, temperature, and power level affect each cycle's contribution to lifetime degradation.
Calendar Life: Number of years the system operates before degrading beyond application requirement. Independent of cycle life.
Safety
Storing large amounts of energy in a confined space leads to a number of safety risks including fire, chemical, electrical, or physical hazards. Different technologies will have their own inherent safety risks or benefits depending on the mechanisms used to store energy. The safety of a system depends heavily upon proper planning and integration early on in a storage project. For help assessing fire risks, please refer to the ESIC Fire Hazard Mitigation Analysis, it is free to the public.
Survey of Technologies
Storage technologies can be grouped into a few categories based on the mechanism that is used to convert and store energy. The categories, with prominent examples, include:
Electrochemical (i.e. Batteries) | Electromechanical | Chemical | Electrical | Thermal |
---|---|---|---|---|
|
|
|
|
|
Below is a table of prominent storage technologies, click on each for more detail.
Storage Technology | AC Efficiency Range | Life | Maturity Level / TRL | Benefits | Challenges | Installed Capacity |
---|---|---|---|---|---|---|
Pumped Hydroelectric Energy Storage | 70-85% | 60-100 years1 | 9 - Fully Mature |
|
| >160 GW |
Compressed Air Energy Storage (CAES) | 40-55% | 30 years | 9 - Fully Mature |
|
| ~500 MW |
Thermal Energy Storage - End Use | Not applicable | 10 - 15 years | 9 - Fully Mature |
|
| Cooling: ~14 GWh2 |
Lithium Ion Batteries | 80-92% | 3,000 - 10,000 cycles 10 - 20 years | 9 - Deployed |
|
| >10 GW |
Flow Batteries | 50-75% | 20 years, >100,000 cycles (claimed) | 8 - deployed (for Vanadium redox). Early deployment / continued R&D. |
|
| ~100 MW |
Flywheel Energy Storage | 85-90% | >100,000 cycles | 7 - Deployed |
|
| ~60 MW |
Thermal Energy Storage - Generation | 35-60% | 20 - 30 years | 4 to 9 - Varied |
|
| ~4 GW (mostly molten salt) |
1"The world’s water battery: Pumped hydropower storage and the clean energy transition", IHA, December 2018 2"Global building and district cooling capacity", IRENA
Research and Resources at EPRI
Current Research Focus
- Long duration storage
- Non-lithium storage
- Lithium ion advancements
Resources and Engagement Opportunities
Resource | Access Level |
---|---|
Webcast Recording on Energy Storage Technology | Publicly Available |
Emerging Energy Storage Technology Testing and Demonstration Supplemental Project | Supplemental Funders |
Energy Storage Technology Database | Program 94: Energy Storage and Distributed Generation or
Program 66: Advanced Generation and Bulk Energy Storage |
Strategic Intelligence (SI) Articles | Program 94: Energy Storage and Distributed Generation |
DER Forum: Technologies Discussion | Program 94: Energy Storage and Distributed Generation |
Energy Storage Integration and Deployment
There are many things that must be considered to successfully deploy an energy storage system. These include:
- Storage Technology Implications
- Exploring technology tradeoffs: Performance, efficiency, materials
- Understanding trends: Cost, performance, maturity
- Balance-of-Plant
- Establishing requirements for auxiliary components
- Understanding impacts on performance, cost, reliability, safety, and environment
- Grid integration
- Standardizing the interface with the grid
- Modeling and analyzing the benefits and costs of storage
- Communications and Control
- Defining interoperable protocols
- Developing operational and dispatch algorithms
- Storage Installation
- Ensuring clean, safe, reliable operation
- Understanding safety and environmental issues
- Developing protocols for operations and maintenance, and for disposal at end of life
- Training and education to make storage a part of the electric power enterprise
Project Lifecycle
The following sections are excerpts from the ESIC Energy Storage Implementation Guide[11][12] which is free to the public. The full report includes a more detailed discussion of these topics.
Planning
Planning describes the process for identifying grid needs, translating such needs into technical requirements, and analyzing the cost-effectiveness and viability of energy storage projects.
Define Grid Need: The first phase in the planning process for an energy storage procurement is the identification of grid needs to characterize applications and services. From the perspective of an electric utility stakeholder, there are several ways energy storage could be used to minimize, defer, or avoid costs; to increase reliability; or to increase the operational efficiency of the electric power system. In addition, there are emerging drivers resulting from the adoption of renewable generation.
Specify Minimum Requirements: Identifying and assessing specific requirements of storage will ensure that project managers evaluate and screen the energy storage technology’s ability to meet solution requirements defined previously.
Technical and Economic Analysis: At this part of the planning phase, there could be multiple options to meet the grid need, including conventional options. The decision makers may narrow the scope of options using a screening cost–benefit analysis. This analysis may include secondary service benefits and costs, in addition to those associated with solving the primary service. Processes such as net market value, a metric that considers the net costs and net benefits of a new generator, and tools such as the Electric Power Research Institute (EPRI) Storage Valuation Estimation Tool (StorageVET) product, a cloud-based energy storage valuation analysis tool, help planners perform analysis on the initial cost-effectiveness screen of the options available.
Project Specific Requirements: Elements for developing energy storage specific project requirements include ownership of the storage asset, energy storage system (ESS) performance, communication and control system requirements, site requirements and availability, local constraints, and safety requirements.
Procurement
Procurement describes the phase of the project that turns previously defined minimum requirements from the planning stage into specifications that result in a formal request for proposal (RFP) or request for offer (RFO). The project team will then review the ESS proposals accordingly and evaluate how well individual proposed systems can meet project needs.
Develop RFP: A significant portion of developing an RFP is the development of the scope of work for the project. The scope of work is the process in which the utility, or the buyer, has the opportunity to define the objectives of the project and include specifications of the ESS, the energy storage product, balance of system, and other physical components and services that are required for the complete integration of the project. It should also clearly describe the expected responsibilities of each party for procuring, designing, and installing different components in the project. To clearly delineate responsibilities and interactions within each task, a division of responsibility (DOR) matrix should be a key supplement to the RFP. Additional ESIC guides and tools to support the development and clear communication of RFP requirements include the ESIC Energy Storage Request for Proposal Guide[13], the ESIC Energy Storage Cost Tool and Template[14], the ESIC Energy Storage Technical Specification Template[15], and the ESIC Energy Storage Safety Guide[16].
Review Proposals and Due Diligence: Proposal responses may include a broad spectrum of potential technologies, configurations, and potentially even supplemental value streams in addition to the core solution being sought by the utility. A well-formed RFP with established criteria for evaluating proposals can simplify the proposal review process. For example, use of the ESIC Energy Storage Technical Specification Template allows the buyer to evaluate and compare technical specifications from potential bidders by requesting the same set of technical information within the same reporting format. Other evaluation criteria may include cost, prior deployment experience, financial stability, and other risk mitigation considerations.
Deployment and Integration
Deployment and Integration describes the stage after procurement contracting has been done until the project has been installed and commissioned, and subsequently handed off to operations. Because energy storage technologies are still emerging, the scope of deployment and integration has not always been fully considered in previous stages. To improve the estimates of time and cost required for implementation, it is important to address in detail the steps required at this stage.
Engineering: Site and system engineering will use contract technical specification requirements and utility and industry design codes and standards as the basis of design.
Permitting: It is important to engage local authorities having jurisdiction (AHJs) to understand permitting requirements and additional codes and standards applicable for the construction and operation of an energy storage system. Due to large gaps in standards for energy storage with respect to codes, standards, and regulations (CSRs) and the lag time for AHJs adopting new CSRs, there may be a need to educate and discuss concerns and requirements for safety, nuisance, or environmental issues with certain departments within an AHJ.
Site Preparation and Construction: After a permit or notice to proceed with construction is issued, site preparation and construction can begin.
Product Manufacture and FAT: In parallel with detailed engineering and site preparation, the energy storage product will be manufactured. When the product manufacturing is complete, it is a common practice for the utility or a third party to witness a factory acceptance test (FAT) at the vendor’s manufacturing facility prior to shipment. The FAT is typically a set of quality control–related tests to help ensure that the components of the ESS have been built to specifications before leaving the factory.
Shipping and Receiving After the ESS is approved for shipment, it is transported to the site. Product shipping and receiving procedures, loading and unloading equipment and practices, modes of transportation, and other considerations should be given careful thought. Responsibility for these tasks should be defined during procurement.
Installation: ESS product installation and system integration can be performed by an electrical contractor who should be experienced in both high- and low-voltage systems and familiar with the local electric utility’s system. However, they may be unfamiliar with energy storage technology and require sufficient training and documentation to ensure that the contractor knows about safety hazards and procedures unique to ESSs.
Commissioning: After the installation and connection of an ESS to the distribution system, commissioning is required to ensure successful integration. The ESIC Energy Storage Commissioning Guide[17] provides details of commissioning and site acceptance tests during the deployment and integration phase.
Interconnection: Before the ESS is allowed to interconnect with the grid, tests and documentation may be required to ensure compliance with interconnection standards.
Acceptance Tests: Additional tests, such as performance and control functionality tests, may be required to verify that the system operates as expected. The ESIC Energy Storage Test Manual[18], with its detailed test protocols that include measurement and calculation methodology, testing duty cycles, and templates for data collection, can be used for acceptance testing.
Operations and Maintenance
Operations and Maintenance (O&M) provides an overview of the various processes steps required over the operational life of the system. Because many of the planning assumptions for the project may evolve over time, it is important to consider both current and future needs while assessing and communicating the inherent strengths and limitations of energy storage technology.
Handoff to Operators: During handoff, it is important that the distribution system and energy resource operators (and other parties with control of storage system) are well-informed and trained regarding the storage system operational software, the intended use of the product, the protection systems and schemes invoked, the planned operational profile of the storage system, and the safety plan.
Maintenance: Maintenance of any asset comes in two forms: planned and unplanned. Planned maintenance should be scheduled regularly, depending on configuration, usage, updates to firmware, and the technology of the ESS, with regular diagnostic checks for indicating degradation and performance expectations. Although costly, unplanned maintenance is needed when storage system malfunctions occur, which may lead to power system reliability issues. Many instances of unplanned maintenance should be avoidable through planned maintenance and diagnostics on system state of health.
Environmental and Safety Reporting: Depending on the type and size of the storage system used, there may be an ongoing requirement to report chemical content, operational status, and other parameters to AHJs.
Update Operational Needs: If changes in operation needs are identified, modeling and simulation efforts may help to understand both the future demand and the current operating needs of the system for energy storage projects. Changes in operation outside the warranty provision or agreed-upon use case may need to be discussed with the vendor or supplier.
Recommissioning: Situations involving replacement of major system components, which could include firmware updates, and changes in operation outside the original scope, may require recommissioning of the system. In addition to recommissioning, periodic performance testing may be conducted to ensure compliance with warranty or to document performance over time.
Decommissioning
Decommissioning and End of Life describes the consideration of issues during the last phase of the project lifecycle when the system is no longer viable. The end of life can be expected by a predetermined project end date, triggered by safety or reliability issues, or caused by exceeding marginal costs relative to marginal benefit. A well-defined end-of-life condition for the energy storage project can ensure the safety, reliability and cost-effectiveness of the project.
Decommissioning: The cost and specifications of decommissioning should be considered throughout all phases of the life cycle. When the decision to decommission an ESS is made, a comprehensive decommissioning plan should be prepared to ensure a safe, efficient process.
Recycling or Disposal: As part of the decommissioning plan, it should be determined what will happen to the system after it leaves the site. A plan could be made to recycle and dispose of the system components or, if there are components that have useful life, they could be reused at another location.
Safety
Energy storage safety should be considered across the entire project lifecycle. Hazards and situations that require more dedicated planning and execution to maintain safe operations should be identified and reviewed often.
Safety Challenges
Key challenges include:
- Varied codes and standards; Adoption takes time
- Misinformation about fire safety hazards still encountered
- Benchmarks for comparison between technologies and products are difficult to find
- Disparate perspectives of diverse stakeholders expose gaps in responsibility
- Lagging communication and education of stakeholders
Safety Codes and Standards
Standard | Description | Recent Edition |
NFPA 1 Fire Code | Chapter 52 includes ESS installation requirements (spacing, fire suppression, ventilation) | 2018 |
NFPA 70 National Electrical Code | Article 706 Energy Storage Systems | 2020 |
IFC 2021 Fire Code | 2018 version had new chapter on energy storage – 2021 is supposed to align with NFPA 855 | Under development |
UL 9540 Energy Storage Systems and Equipment | Product safety standard for an ESS: system level; References numerous other standards | 2020 |
UL 9540a Fire Safety Testing Protocol | Provides test procedure for fire safety and thermal runaway propagation | 2019 |
IEEE C2-17 National Electric Safety Code (NESC) | New material inserted relating to Storage Safety | 2017 |
NFPA 855 Standard for the Installation of Stationary Energy Storage Systems |
|
2020 |
References
- ↑ Helman U, Kaun B, and Stekli J (2020) "Development of Long-Duration Energy Storage Projects in Electric Power Systems in the United States: A Survey of Factors Which Are Shaping the Market"
- ↑ "US DOE Global Energy Storage Database"
- ↑ "2024 World Hydropower Outlook"
- ↑ BloombergNEF: "A Behind the Scenes Take on Lithium-ion Battery Prices"
- ↑ "EPRI Battery Energy Storage Lifecyle Cost Assessment Summary: 2020"
- ↑ California Independent System Operator (CAISO): "What the duck curve tells us about managing a green grid"
- ↑ Lawrence Berkeley National Lab, Hybrid Power Plants: Status of Installed and Proposed Projects
- ↑ Pacific Northwest National Laboratory (PNNL): Energy Storage Policy Database
- ↑ EPRI: "Battery Energy Storage Lifecycle Cost Assessment Summary 2020"
- ↑ EPRI: "Battery Energy Storage Lifecycle Cost Assessment Summary 2020"
- ↑ EPRI Energy Storage Integration Council (ESIC) Website
- ↑ ESIC Energy Storage Implementation Guide
- ↑ ESIC Energy Storage Request for Proposal Guide
- ↑ ESIC Energy Storage Cost Tool and Template
- ↑ ESIC Energy Storage Technical Specification Template
- ↑ ESIC Energy Storage Safety Guide
- ↑ ESIC Energy Storage Commissioning Guide
- ↑ ESIC Energy Storage Test Manual
Additional Resources